When a star the size of 20 suns dies, it becomes, in the words of astrophysicist Zaven Arzoumanian, “the most outrageous object that most people have never heard of”—a city-size body of improbable density known as a neutron star. A chunk of neutron star the size of a Ping-Pong ball would weigh more than a billion metric tons. Below the star’s surface, under the crush of gravity, protons and electrons melt into one another to form a bulk of mostly neutrons—hence the name. At least, that is what we think. The issue is far from settled. Astronomers have never seen a neutron star up close, and no laboratory on Earth can create anything even approaching the same density, so the inner structure of these objects is one of the greatest mysteries in space. “They are matter at the highest stable density that nature allows, in a configuration that we don’t understand,” says Arzoumanian, who works at NASA’s Goddard Space Flight Center. They are also the most strongly gravitating form of matter known—add just a bit more mass, and they would be black holes, which are not matter at all but rather purely curved space. “What goes on at that threshold,” Arzoumanian says, “is what we’re trying to explore.”
There are several competing theories about what goes on at that threshold. Some ideas suggest that neutron stars really are just full of regular neutrons and maybe a few protons here and there. Others propose much stranger possibilities. Perhaps the neutrons inside neutron stars dissolve further into their constituent particles, called quarks and gluons, which swim untethered in a free-flowing sea. And it is possible that the interiors of these stars are made of even more exotic stuff, such as hyperons—weird particles composed not of regular “up” and “down” quarks (the kind found in atoms) but their heavier “strange quark” cousins.
Short of cutting open a neutron star and looking inside, there is no easy way to know which of these theories is right. But scientists are making progress. A big break came in August 2017, when terrestrial experiments detected gravitational waves—undulations in spacetime produced by the acceleration of massive objects—from what looked like a head-on collision of two neutron stars. These waves carried information about the masses and sizes of the stars right before the crash, which scientists have used to place new limits on the properties and possible compositions of all neutron stars.
On supporting science journalism
If you're enjoying this article, consider supporting our award-winning journalism by subscribing. By purchasing a subscription you are helping to ensure the future of impactful stories about the discoveries and ideas shaping our world today.
Clues are also coming from the Neutron Star Interior Composition Explorer (NICER), an experiment that started at the International Space Station in June 2017. NICER watches pulsars, which are highly magnetic, furiously rotating neutron stars that emit sweeping beams of light. As these beams pass over Earth, we see pulsars blink on and off at more than 700 times a second. Through these experiments and others, the prospect of understanding what is inside a neutron star finally looks possible. If scientists can do that, they will have a handle not just on one class of cosmic oddity but on the fundamental limits of matter and gravity as well.
Superfluid seas
Neutron stars are forged in the cataclysms known as supernovae, which occur when stars run out of fuel and cease generating energy in their cores. Suddenly gravity has no opposition, and it slams down on the star like a piston, blowing the outer layers away and smashing the core, which at this point in a star’s life is mostly iron. The gravity is so strong it quite literally crushes the atoms, pushing the electrons inside the nucleus until they fuse with protons to create neutrons. “The iron is compressed by a factor of 100,000 in each direction,” says Mark Alford, a physicist at Washington University in St. Louis. “The atom goes from being a tenth of a nanometer across to just a blob of neutrons a few femtometers wide.” That is like shrinking Earth down to the size of a single city block. (A femtometer is a millionth of a nanometer, which is itself a billionth of a meter.) When the star has finished collapsing, it contains about 20 neutrons for every proton. It is much like a single giant atomic nucleus, says James Lattimer, an astronomer at Stony Brook University—with an important difference. “A nucleus is held together by nuclear interactions,” Lattimer says. “A neutron star is held together by gravity.”
Astronomers Walter Baade and Fritz Zwicky proposed neutron stars in 1934 as an answer to the question of what might be left over after a supernova—a term they coined at the same time for the extra-bright explosions being spotted across the sky. It had only been two years since British physicist James Chadwick discovered the neutron. Initially some scientists were skeptical that such extreme objects could exist, and it was not until Jocelyn Bell Burnell and her colleagues observed pulsars in 1967—and researchers over the next year determined they must be spinning neutron stars—that the idea was widely accepted.
Physicists think that neutron stars can range from roughly one to two and a half times the mass of the sun and that they probably consist of at least three layers. The outer layer is a gaseous “atmosphere” of hydrogen and helium a few centimeters to meters thick. It floats atop a kilometer-deep outer “crust” made of atomic nuclei arranged in a crystal structure, with electrons and neutrons between them. The third, interior layer, which makes up the bulk of the star, is a bit of a mystery. Here nuclei are crammed in as tight as the laws of nuclear physics will allow, with no separation between them. As you move inward toward the core, each nucleus holds ever larger numbers of neutrons. At some point, the nuclei cannot contain any more neutrons, so they spill over: now there are no nuclei anymore, just nucleons (that is, neutrons or protons). Eventually in the innermost core, these may break down as well. “We are in the hypothetical regime where we do not know what happens at these insane pressures and densities,” Alford says. “What we think might happen is that the neutrons actually get crushed together, and they overlap so much you can’t really talk about it as being a fluid of neutrons anymore but a fluid of quarks.”
What form that fluid takes is an open question. One possibility is that the quarks form a “superfluid,” which has no viscosity and, once set in motion, will theoretically never stop moving. This bizarre state of matter is possible because quarks feel an affinity for other quarks, and if they are pushed close enough together, they can form bound “Cooper pairs.” By itself, a quark is a fermion—a particle whose spin has the quantum-mechanical value of half an integer. When two quarks pair up, together they act as a single boson—a particle with spin equal to zero or one or another integer. After this change, the particle follows new rules. Fermions are bound by the Pauli exclusion principle, which says that no two identical fermions can occupy the same state—but bosons have no such restrictions. When they were fermions, the quarks were forced to take on higher energies to stack on top of one another in the crowded neutron stars. As bosons, however, they can stay in the lowest-possible energy state—any particle’s preferred position—and still cram in together. When they do this, the quark pairs form a superfluid.
Outside the densest part of the core, where neutrons are likely intact, neutrons can also pair up to make a superfluid. In fact, scientists are fairly sure neutrons in the crust of the star do this. The evidence comes from observations of pulsar “glitches,” episodes in which a spinning neutron star rapidly speeds up. Theorists think that these glitches occur when the rotation speed of the star as a whole grows out of sync with the rotation of the superfluid inside its crust. Overall, the star’s rotation naturally slows with time; the superfluid, flowing without friction, does not. When the difference between these rates gets too great, the superfluid transfers angular momentum to the crust. “It’s like an earthquake,” Lattimer says. “You get a hiccup and a burst of energy, and the spin frequency increases for a brief time and then settles back down again.”
In 2011 Lattimer and his colleagues suggested they had also found evidence of a superfluid in a neutron star’s core, but he admits that this is still open to debate. To find that evidence, Lattimer’s team, led by Dany Page of the National Autonomous University of Mexico, studied 15 years of x-ray observations of Cassiopeia A, the remnant of a supernova that first became visible on Earth in the 17th century. The scientists found that the pulsar at the center of the nebula is cooling faster than traditional theory suggests it should. One explanation is that many of the neutrons inside the star are pairing up to become a superfluid. The pairs break and re-form, emitting neutrinos, which causes the neutron star to lose energy and cool off. “This is something we never thought we would see,” Lattimer says. “But lo and behold, there is this one star with the right age for us to see this. The proof in the pudding is going to come in another 50 or so years, when it should start to cool more slowly because once the superfluid is made, there is no more extra energy to be lost.”
Weird quarks
Superfluids are only one of the exotic possibilities waiting behind the mystery doors of neutron stars. It is also possible that they are home to rare “strange quarks.”
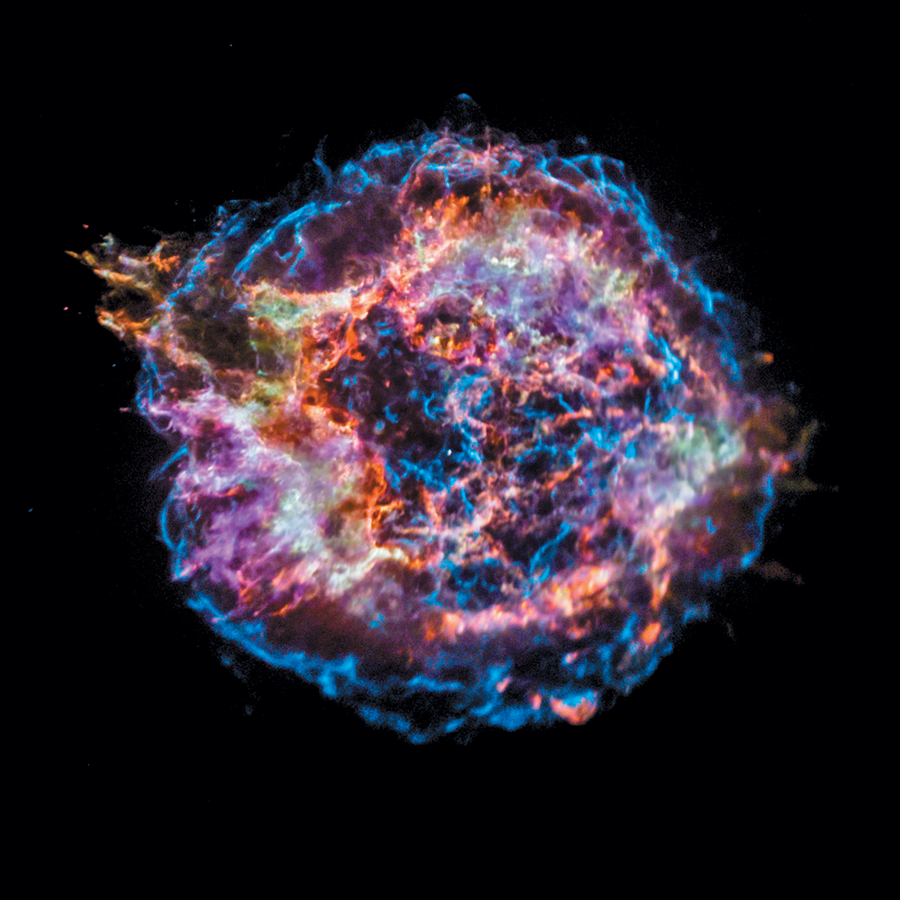
CASSIOPEIA A is the remnant of an ancient supernova. At its center is a neutron star whose core may contain “superfluid.” Credit: NASA, CXC and SAO
Quarks come in six kinds, or flavors—up, down, charm, strange, top and bottom. Only the lightest two, up and down, are found in atoms. The rest of the flavors are so massive and unstable that they usually appear only as short-lived detritus from high-energy particle collisions inside atom smashers such as the Large Hadron Collider at CERN near Geneva. But in the extremely dense interior of neutron stars, the up and down quarks inside neutrons might sometimes transform into strange quarks. (The other unusual flavors—charm, top and bottom—are so massive that they likely would not form even there.) If strange quarks appear and remain bound to other quarks, they would make the mutant neutrons called hyperons. It is also possible that these quarks are not contained in particles at all—they might roam freely in a kind of quark soup.
Each of these possibilities should change the size of neutron stars in a measurable way. Intact neutrons inside the core would, in Arzoumanian’s words, act “like marbles and make a hard, solid core.” The solid core would tend to push on the outer layers and increase the size of the entire star. On the other hand, if the neutrons dissolved into a stew of quarks and gluons, they would make a “softer, squishier” and smaller star, he says. Arzoumanian is a co-principal investigator and science lead for the NICER experiment, which aims to determine which of these alternatives is true: “One of NICER’s key objectives is to make a measurement of [neutron stars’] mass and radius that will help us pick out or exclude certain theories of dense matter.”
NICER is a washing-machine-size box mounted to the exterior of the International Space Station. It steadily monitors several dozen pulsars spread across the sky, detecting x-ray photons from them. By measuring the photons’ timing and energy, as well as how the stars’ gravitational fields bend their light, NICER allows scientists to calculate the masses and radii of a collection of pulsars and compare them. “If NICER finds stars with roughly the same mass but very different radii, that would mean there’s something funny going on,” Alford says, “some new form of matter that, when it appears, makes the stars shrink down.” Such a transition could occur, for instance, when neutrons break apart into quarks and gluons.
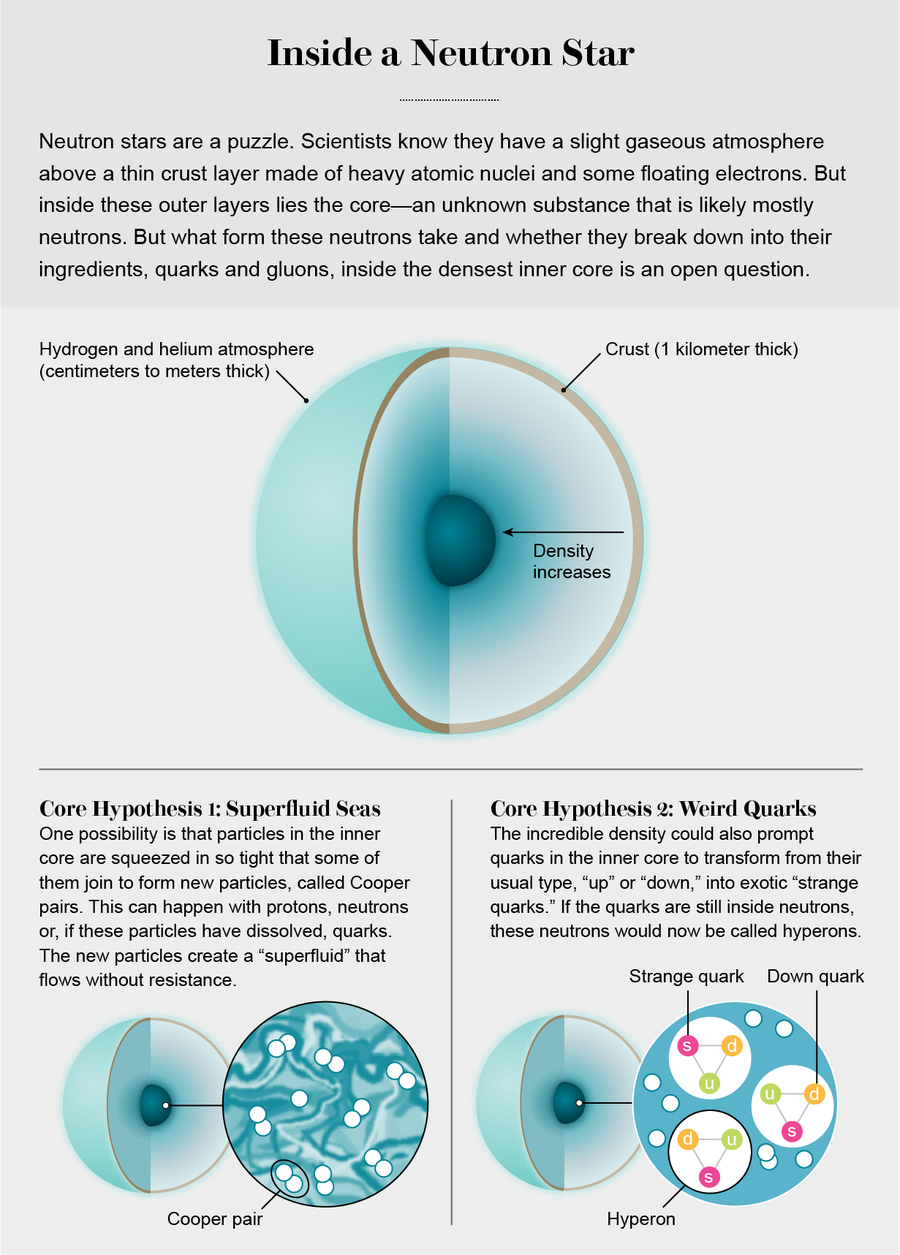
Credit: Nigel Hawtin
Measuring the sizes of neutron stars is a useful way to narrow the range of possible forms that matter inside neutron stars can take. Scientists once thought half the neutrons in any given neutron star would turn into hyperons that contained strange quarks; theoretical calculations suggested that such a hyperon-rich star could not exceed 1.5 times the mass of the sun. In 2010, however, astronomers led by Paul Demorest of the National Radio Astronomy Observatory measured the mass of one neutron star at 1.97 solar masses, eliminating a number of theories about the interior of a neutron star. Now physicists estimate that hyperons cannot make up more than 10 percent of a neutron star.
Crash site detectives
Studying individual neutron stars can tell us a lot, but we can learn much more when two of them slam together. For years telescopes have detected blasts of light, called gamma-ray bursts, that researchers suspected came from a crash of two neutron stars. In the August 2017 detection of gravitational waves, astronomers saw the first confirmed neutron star merger.
Specifically, on August 17, 2017, two experiments—the Laser Interferometer Gravitational-wave Observatory, or LIGO (based in Washington State and Louisiana), and Virgo (a European project based near Pisa, Italy)—simultaneously detected gravitational ripples produced as two neutron stars spiraled toward each other and merged to form either a single neutron star or a black hole. This was not the first detection of gravitational waves, but all the previous sightings were created by the collisions of two black holes. Before this date, scientists had never observed waves coming from neutrons stars, and this was also the first time that telescopes had responded to a gravitational-wave detection and seen light coming from the same place in the sky at the same time. The light and waves together provided a bounty of information about where and how the crash happened that proved a boon for neutron star physics. “I was quite flabbergasted,” Lattimer says of the lucky observation. “I thought this is just too good to be true.”
Astrophysicists traced the waves back to a pair of neutron stars about 130 million light-years from Earth. The details of the waves—their frequency and strength and the pattern they followed over time—allowed researchers to estimate that each weighed roughly 1.4 solar masses and stretched between 11 and 12 kilometers in radius before the crash. This knowledge will help scientists to formulate an essential descriptor for understanding neutron stars—their equation of state. The equation describes the density matter will take under different pressures and temperatures and should apply to all neutron stars in the universe. Theorists have come up with several possible formulations of the equation of state that correspond to different configurations of matter inside neutron stars, and the new measurements offered a chance to rule some out. The discovery that the neutron stars’ radii were relatively small, for instance, was a surprise. Some theories run into difficulty when they try to fit both these compact neutron stars and known heavy stars, such as the 1.97-solar-mass behemoth, into the same fundamental equation of state. “It’s starting to make our equation of state thread a needle path through these different observations,” says Jocelyn Read, an astrophysicist at California State University, Fullerton, and co-leader of LIGO’s Extreme Matter team. “Trying to make compact stars, as well as supporting massive stars, is getting to be challenging to the theory. It’s definitely interesting and might get more interesting.”
So far LIGO and Virgo have seen only this one neutron star collision, but another such observation could come any day now. “I’ve been working in this field long enough,” Read says, “that it’s just so fantastic to move from an era of what-ifs: ‘If we could see gravitational waves, then we might be able to do this.’ Now we’re actually getting a chance to do this, and it hasn’t gotten old yet.”
The limits of matter
In time, as gravitational-wave detectors improve in sensitivity, the payoffs could be huge. For instance, one test of what is inside a neutron star involves looking for gravitational waves emitted by any swirling liquid in its middle. If the liquid has very low or no viscosity—as a superfluid would—it might begin flowing in patterns, called r-modes, that release gravitational waves. “These gravitational waves would be much weaker than from a merger,” Alford says. “It is matter quietly sloshing versus being ripped apart.” Alford and his collaborators determined that the currently running Advanced LIGO detector would not be able to see these waves, but future upgrades to LIGO, as well as planned observatories such as the ground-based Einstein Telescope under consideration in Europe, might.
Cracking the case on neutron stars would give us a picture of matter at its barely comprehensible extremes—a form so removed from the atoms that make up our world that it stretches the bounds of what is possible. It might turn imagined curiosities such as sloshing quark matter, superfluid neutrons and outlandish hyperon stars into reality. And understanding neutron stars could do something more: physicists’ deeper goal is to use these squashed stars to tackle larger open questions, such as the laws that govern nuclear interactions—the complicated dance among protons, neutrons, quarks and gluons—as well as the biggest mystery of all—the nature of gravity.
Neutron stars are just one way of investigating nuclear forces, and simultaneous work is going on at particle accelerators around the world, which act like microscopes to peer inside atomic nuclei. When more of the nuclear problem is nailed down, scientists can turn their focus to gravity. “Neutron stars are a mixture of gravitational physics and nuclear physics,” says Or Hen, a physicist at the Massachusetts Institute of Technology. “Right now we are using neutron stars as a lab to understand nuclear physics. But because we have access to nuclei here on Earth, we should be able to constrain the nuclear aspect of the problem well enough eventually. Then we can use neutron stars to understand gravity, which is one of the biggest challenges in physics.”
Gravity as currently understood—through Einstein’s general theory of relativity—does not get along with the theory of quantum mechanics. Ultimately one of the theories must budge, and physicists do not know which it will be. “We will get there,” Hen says, “and that is a very exciting prospect.”